Material Limits Impact Infrastructure Plans
Material Limits Impact Infrastructure Plans
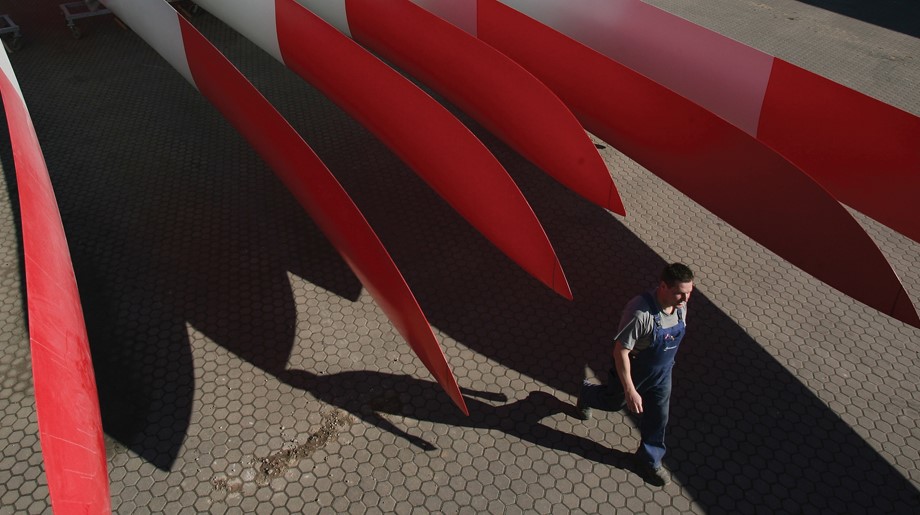
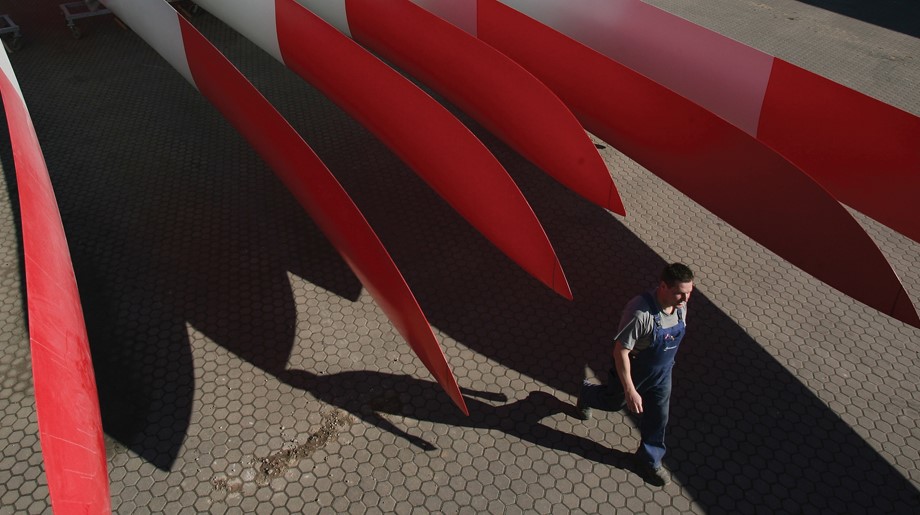
Any buildout of new energy infrastructure will run headlong into production constraints for concrete, copper, and other critical materials.
One of the greatest challenges of this century is the reduction or elimination of worldwide greenhouse gas emissions, primarily by moving the global energy system from carbon-based fuels to low- or zero-carbon systems. It will be a monumental undertaking, and yet the scope, difficulty, and complexity of decarbonizing the global energy supply have been widely, and repeatedly, underestimated.
For instance, although the process of decarbonization has been explicitly underway for more than two decades, the world has been getting more, not less, dependent on fossil carbon every year. At present, it is impossible to identify the eventual composition of carbon-free energy supply: What shares of electric generation will come from wind, solar, and fission? How many vehicles will run on fuel cells or green ammonia rather than on batteries? The eventual costs of this global undertaking are impossible to estimate with satisfactory accuracy, but they will total many hundreds of trillions of dollars, implying the need to spend—every year, for decades to come—a significant share of the global economic product (now about $100 trillion) on the quest.
While the cost alone is daunting, recent years have seen a belated concern about another fundamental challenge: the enormous material needs required by decades of energy transition. Those needs will include replacing more than 4 TW of installed fossil-fueled electricity-generating capacity (now concentrated in large central stations) by non-carbon conversion by wind turbines, PV cells, or nuclear reactors; substituting 1.4 billion combustion (gasoline and diesel) engines on the road by batteries or by non-fossil fuels; finding new ways to smelt iron ores without one billion tons of coal-derived coke; replacing more than half a billion natural gas furnaces (in houses and in industrial and commercial establishments) by heat pumps or other sources of heat; and introducing new non-carbon feedstocks to synthesize more than 400 million tons of plastics and nearly 200 million tons of ammonia.
Become a Member of ASME
The economic shift in recent decades from manufacturing to services might suggest this transition could be accomplished via dematerialized or even virtual means. For many people, downloaded digital files have replaced physical books, newspapers, and music recordings, and working from home substitutes for commuting to an office. But the energy systems that produce carbon emissions are inherently physical and must be replaced by other physical systems, which means the material constraints of building this new infrastructure cannot be ignored.
To put that number into perspective, in 2022 wind and PV generation contributed less than 15 percent of the world’s electricity supply.
Wind may be considered a more attractive option for expansion than photovoltaics, given that wind has a capacity factor two to four times that of solar and installations costs per MW have been declining as typical sizes of wind turbines have been increasing. (The largest offshore units now in operation rate 14 MW and 16 MW.) If wind were to generate two-thirds of the projected renewable electricity supply then even with high average capacity factor of 35 percent (thanks to massive offshore wind farms set in more windy environments) that would require about 9 TW of additional wind power —compared to 906 GW in 2021, almost exactly an order of magnitude increase in 27 years.
Wind power projects require a variety of materials: concrete and reinforcing steel for the turbines’ massive foundations; tubular steel for their towers; epoxy resins lightened with balsa and carbon fibers for their three long blades; steel alloys, aluminum, and plastics for their aerodynamically shaped nacelles that house turbines’ mechanical and electrical components made largely of steel, copper, and aluminum, and two rare metals, neodymium and praseodymium, for their permanent magnets. Specific material needs, measured in tons per MW of generating capacity, vary with turbine capacity (declining with size) and location (higher for massive offshore unit anchored in relatively deep water) but aggregate needs for typical units (including their concrete and reinforced steel foundations) are about 500 tons per MW.
More on Manufacturing: Electric Vehicle Makers Work Out Supply Chain Kinks
As expected, concrete dominates, accounting for about 70 percent of the mass. Metals—dominated by unalloyed and alloyed steel, with lesser amounts of cast iron, aluminum, and copper—provide another 27 percent, while the rest is made up of polymers, balsa, and carbon fibers (to lighten the blades), ceramics, rare earths, electronics, and lubricants. This means that installing 9 TW of new wind capacities between 2023 and 2050 would require more than 3 billion tons of concrete and 1.2 billion tons of metals (mostly steel): the former total is equal to about two-thirds of annual global production, the latter is almost exactly equal to annual output of primary (blast furnace) iron.
These material requirements look particularly large when wind turbines are compared with gas turbines, now the most efficient, the most compact, and the most flexible machines for electricity generation. Specifications by Siemens, the leading producer of gas turbines, show that a complete installation of machines in the 40 MW to 50 MW range—relatively small ones, as the largest units are more than 10 times larger—requires between 250,000 kilograms and 290,000 kilograms of material for the turbine itself plus the associated power generation and drive packages. That equals about 6 tons of material per MW, and even if we quintuple that rate to account for foundations, enclosing structure, connections to a pipeline and venting, the aggregate of some 30 tons per MW would be just 6 percent of a wind turbine’s material demand.
As a result, large-scale accelerated production and installation of wind turbines would bring a temporary increase in fossil fuel demand and in associated greenhouse gas emissions amounting to about 7 grams of CO2 equivalent for every generated kWh. To be sure, this bump is only temporary as the paybacks for both the energy embedded in turbines and CO2 emissions attributed to their materials are, depending on turbine size and location, just six to 12 months: Afterward, and for the rest of their lifetimes, the machines are generators of carbon-free electricity.
But wind generation is just a part of a larger material challenge associated with rapid decarbonization. Other demands are even more challenging because of the need for metallic ores that contain steadily lower concentrations of the needed elements.
Copper, indispensable for electric vehicles, is a prime example of these consequences. The average EV contains about 80 kg of it, compared to less than 15 kg in an internal combustion engine car. Replacing today’s 1.4 billion ICE vehicles by EVs would thus require more than 90 million tons of additional copper supply during the next 27 years. Copper extraction for all purposes was about 21 million tons in 2022.
Watch the Video: Electric Vehicles Reshape the Automotive Industry
But this comparison hides the enormous displacement of waste solids and high energy expenditures associated with this demand. While hematite, the most common iron ore, has up to 60 percent iron and bauxite has 15 percent to 25 percent aluminum, the dominant copper ores exploited in the early 2020s have only 0.6 percent of the metal, and further quality decline is inevitable. This means that the extraction of additional 90 million tons of copper by 2050 would require drilling, blasting, removal, processing, and waste deposition amounting to about 15 billion tons of rock, a mass equivalent to the world’s annual extraction of all fossil fuels and of all metallic ores, combined.
Similarly, if we were to eliminate coke in primary iron smelting and natural gas from ammonia production processes, replacing both with green hydrogen generated using renewable electricity, just these two uses would require new electrolytic processes capable of producing about 90 million tons of H2 a year. That would inevitably run into high supply risks concerning the availability of platinum, iridium, scandium, and yttrium catalyzers, and, of course, it would be predicated on assuring about 5 PWh of uninterrupted supply of green electricity (equivalent of nearly 20 percent of today’s total global generation).
Selected for You: Leading Hard-to-Decarbonize Industries to Zero Emissions
Finally, the International Energy Agency expects that compared to 2020, the expansion of EVs and battery storage could raise the demand for lithium by over 40 times, and those of graphite, cobalt, and nickel by up to 25 times by 2040.
Because we are at the beginning of what will prove to be a long process, annual installation rates of green energy conversions will have to increase substantially and must be sustained over the course of decades. Achieving such progress is fundamentally dependent on the multiplication of global supplies of a wide range of materials, minerals, and elements, ranging from heavy and common (steel and concrete) to common but highly energy intensive (copper, aluminum, and silicon) and to uncommon and strategically important (cobalt, rare earths).
And even raising material production to such highs is no guarantee that green energy projects will receive them. Decarbonization is in inevitable competition with the material needs of other priorities, such as the need to fix aging infrastructures in affluent economies and to build badly needed new ones in low-income countries.
Check Out Our Infographic: Electric Vehicles Need Imported Minerals
Tackling these material challenges also require a focus from engineers to develop more efficient processes and new machines that require fewer or different materials. However, while good engineering can lessen the degree of the material constraints, they can’t erase them altogether.
That last point is the most important one to remember. Some may think that we now live in a dematerialized era, where data is considered the new oil and AI is talked of as the new electricity. However, that would a profound categorical mistake: Matter always matters, never more so than when trying to make the modern world free of fossil carbon.
Vaclav Smil is Distinguished Professor Emeritus at the University of Manitoba in Winnipeg. He is the author of 47 books, including his most recent, Invention and Innovation: A Brief History of Hype and Failure, published in February 2023.
For instance, although the process of decarbonization has been explicitly underway for more than two decades, the world has been getting more, not less, dependent on fossil carbon every year. At present, it is impossible to identify the eventual composition of carbon-free energy supply: What shares of electric generation will come from wind, solar, and fission? How many vehicles will run on fuel cells or green ammonia rather than on batteries? The eventual costs of this global undertaking are impossible to estimate with satisfactory accuracy, but they will total many hundreds of trillions of dollars, implying the need to spend—every year, for decades to come—a significant share of the global economic product (now about $100 trillion) on the quest.
While the cost alone is daunting, recent years have seen a belated concern about another fundamental challenge: the enormous material needs required by decades of energy transition. Those needs will include replacing more than 4 TW of installed fossil-fueled electricity-generating capacity (now concentrated in large central stations) by non-carbon conversion by wind turbines, PV cells, or nuclear reactors; substituting 1.4 billion combustion (gasoline and diesel) engines on the road by batteries or by non-fossil fuels; finding new ways to smelt iron ores without one billion tons of coal-derived coke; replacing more than half a billion natural gas furnaces (in houses and in industrial and commercial establishments) by heat pumps or other sources of heat; and introducing new non-carbon feedstocks to synthesize more than 400 million tons of plastics and nearly 200 million tons of ammonia.
Become a Member of ASME
The economic shift in recent decades from manufacturing to services might suggest this transition could be accomplished via dematerialized or even virtual means. For many people, downloaded digital files have replaced physical books, newspapers, and music recordings, and working from home substitutes for commuting to an office. But the energy systems that produce carbon emissions are inherently physical and must be replaced by other physical systems, which means the material constraints of building this new infrastructure cannot be ignored.
Mass effect
So far, the greatest progress in relative decarbonization of the global energy supply has been overwhelmingly due to reducing carbon intensity of electricity generation. This shift has been driven by widespread installation of new wind turbines and photovoltaic cells and, as with most instances of early expansion, these conversions have seen high annual growth rates. Not surprisingly, all long-range forecasts of decarbonization trajectories foresee very large increases of renewable electricity capacities for decades to come. To cite a typical example, the latest forecast by Enerdata, an independent research company that analyzes forecasts energy and climate developments, assumes that the global electricity consumption will reach about 52 PWh (or 52 million GWh) in 2050—more than 90 percent above the 2022 total—and that renewables will supply 80 percent of that.To put that number into perspective, in 2022 wind and PV generation contributed less than 15 percent of the world’s electricity supply.
Wind may be considered a more attractive option for expansion than photovoltaics, given that wind has a capacity factor two to four times that of solar and installations costs per MW have been declining as typical sizes of wind turbines have been increasing. (The largest offshore units now in operation rate 14 MW and 16 MW.) If wind were to generate two-thirds of the projected renewable electricity supply then even with high average capacity factor of 35 percent (thanks to massive offshore wind farms set in more windy environments) that would require about 9 TW of additional wind power —compared to 906 GW in 2021, almost exactly an order of magnitude increase in 27 years.
Wind power projects require a variety of materials: concrete and reinforcing steel for the turbines’ massive foundations; tubular steel for their towers; epoxy resins lightened with balsa and carbon fibers for their three long blades; steel alloys, aluminum, and plastics for their aerodynamically shaped nacelles that house turbines’ mechanical and electrical components made largely of steel, copper, and aluminum, and two rare metals, neodymium and praseodymium, for their permanent magnets. Specific material needs, measured in tons per MW of generating capacity, vary with turbine capacity (declining with size) and location (higher for massive offshore unit anchored in relatively deep water) but aggregate needs for typical units (including their concrete and reinforced steel foundations) are about 500 tons per MW.
More on Manufacturing: Electric Vehicle Makers Work Out Supply Chain Kinks
As expected, concrete dominates, accounting for about 70 percent of the mass. Metals—dominated by unalloyed and alloyed steel, with lesser amounts of cast iron, aluminum, and copper—provide another 27 percent, while the rest is made up of polymers, balsa, and carbon fibers (to lighten the blades), ceramics, rare earths, electronics, and lubricants. This means that installing 9 TW of new wind capacities between 2023 and 2050 would require more than 3 billion tons of concrete and 1.2 billion tons of metals (mostly steel): the former total is equal to about two-thirds of annual global production, the latter is almost exactly equal to annual output of primary (blast furnace) iron.
These material requirements look particularly large when wind turbines are compared with gas turbines, now the most efficient, the most compact, and the most flexible machines for electricity generation. Specifications by Siemens, the leading producer of gas turbines, show that a complete installation of machines in the 40 MW to 50 MW range—relatively small ones, as the largest units are more than 10 times larger—requires between 250,000 kilograms and 290,000 kilograms of material for the turbine itself plus the associated power generation and drive packages. That equals about 6 tons of material per MW, and even if we quintuple that rate to account for foundations, enclosing structure, connections to a pipeline and venting, the aggregate of some 30 tons per MW would be just 6 percent of a wind turbine’s material demand.
Copper and lithium
Another intractable challenge to building out wind turbines as a decarbonization strategy is that in the foreseeable future, those massive new material needs for wind turbine installation would translate into temporarily higher demand for fossil energies. Today, in the early 2020s, we have no commercially available processes that would allow us to accomplish at large scales these necessary tasks: to smelt billions of tons of primary iron without coal-derived coke that reduces iron ores in blast furnaces; to produce billions of tons of concrete without burning any fossil fuels; to produce tens of millions of tons of rotor plastics without fossil fuel feedstocks; and to supply millions of tons of lubricants required for smooth turbine operation.As a result, large-scale accelerated production and installation of wind turbines would bring a temporary increase in fossil fuel demand and in associated greenhouse gas emissions amounting to about 7 grams of CO2 equivalent for every generated kWh. To be sure, this bump is only temporary as the paybacks for both the energy embedded in turbines and CO2 emissions attributed to their materials are, depending on turbine size and location, just six to 12 months: Afterward, and for the rest of their lifetimes, the machines are generators of carbon-free electricity.
But wind generation is just a part of a larger material challenge associated with rapid decarbonization. Other demands are even more challenging because of the need for metallic ores that contain steadily lower concentrations of the needed elements.
Copper, indispensable for electric vehicles, is a prime example of these consequences. The average EV contains about 80 kg of it, compared to less than 15 kg in an internal combustion engine car. Replacing today’s 1.4 billion ICE vehicles by EVs would thus require more than 90 million tons of additional copper supply during the next 27 years. Copper extraction for all purposes was about 21 million tons in 2022.
Watch the Video: Electric Vehicles Reshape the Automotive Industry
But this comparison hides the enormous displacement of waste solids and high energy expenditures associated with this demand. While hematite, the most common iron ore, has up to 60 percent iron and bauxite has 15 percent to 25 percent aluminum, the dominant copper ores exploited in the early 2020s have only 0.6 percent of the metal, and further quality decline is inevitable. This means that the extraction of additional 90 million tons of copper by 2050 would require drilling, blasting, removal, processing, and waste deposition amounting to about 15 billion tons of rock, a mass equivalent to the world’s annual extraction of all fossil fuels and of all metallic ores, combined.
Similarly, if we were to eliminate coke in primary iron smelting and natural gas from ammonia production processes, replacing both with green hydrogen generated using renewable electricity, just these two uses would require new electrolytic processes capable of producing about 90 million tons of H2 a year. That would inevitably run into high supply risks concerning the availability of platinum, iridium, scandium, and yttrium catalyzers, and, of course, it would be predicated on assuring about 5 PWh of uninterrupted supply of green electricity (equivalent of nearly 20 percent of today’s total global generation).
Selected for You: Leading Hard-to-Decarbonize Industries to Zero Emissions
Finally, the International Energy Agency expects that compared to 2020, the expansion of EVs and battery storage could raise the demand for lithium by over 40 times, and those of graphite, cobalt, and nickel by up to 25 times by 2040.
Engineering focus
The material challenges of the replacing fossil fuels by low- or zero-carbon energy conversions are not insurmountable but tackling them must begin by realizing that the absolute decarbonization of global energy supply is yet to begin: Fossil fuels still account for 82 percent of the world’s primary energy consumption. We are still in the earliest phase of the transition even as far as the decarbonization of electricity generation is concerned.Because we are at the beginning of what will prove to be a long process, annual installation rates of green energy conversions will have to increase substantially and must be sustained over the course of decades. Achieving such progress is fundamentally dependent on the multiplication of global supplies of a wide range of materials, minerals, and elements, ranging from heavy and common (steel and concrete) to common but highly energy intensive (copper, aluminum, and silicon) and to uncommon and strategically important (cobalt, rare earths).
And even raising material production to such highs is no guarantee that green energy projects will receive them. Decarbonization is in inevitable competition with the material needs of other priorities, such as the need to fix aging infrastructures in affluent economies and to build badly needed new ones in low-income countries.
Check Out Our Infographic: Electric Vehicles Need Imported Minerals
Tackling these material challenges also require a focus from engineers to develop more efficient processes and new machines that require fewer or different materials. However, while good engineering can lessen the degree of the material constraints, they can’t erase them altogether.
That last point is the most important one to remember. Some may think that we now live in a dematerialized era, where data is considered the new oil and AI is talked of as the new electricity. However, that would a profound categorical mistake: Matter always matters, never more so than when trying to make the modern world free of fossil carbon.
Vaclav Smil is Distinguished Professor Emeritus at the University of Manitoba in Winnipeg. He is the author of 47 books, including his most recent, Invention and Innovation: A Brief History of Hype and Failure, published in February 2023.
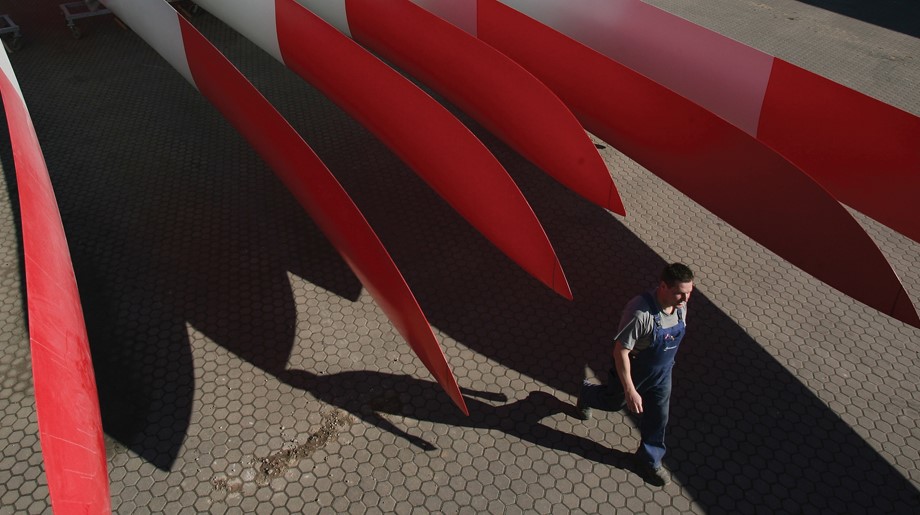
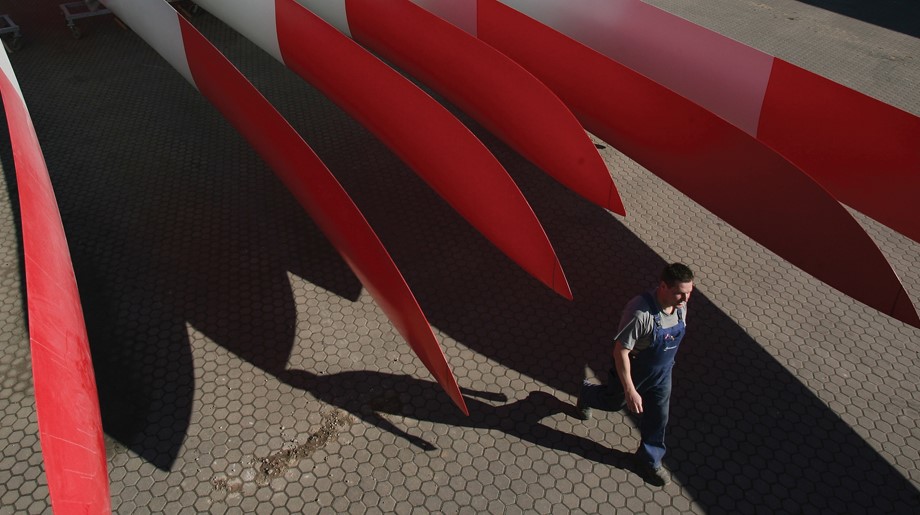