The Model of Good Health
The Model of Good Health
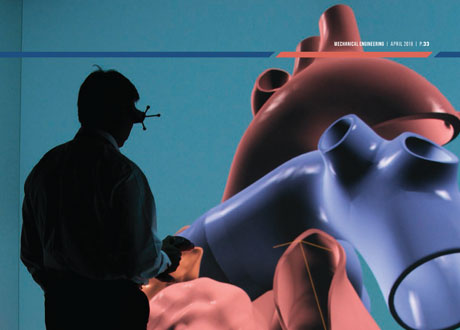
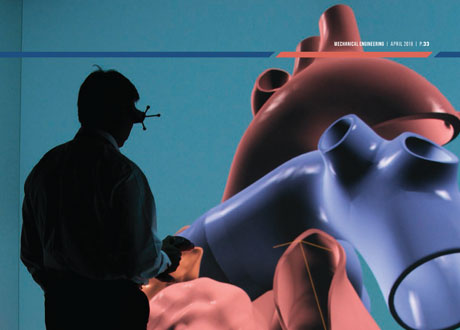
In some ways, medical devices are just another kind of product. Medical devices—everything from prosthetic limbs and artificial hearts to the most prosaic tongue depressors—are designed by engineers to meet customer specifications; they are manufactured with both cost and quality in mind; and they have to compete in the marketplace against similar products from rival companies.
But medical device manufacturers face challenges like no other industry. Each new device has to run a gauntlet of regulatory and reporting demands, and biomedical engineers work with the knowledge that a design mishap could result in death and that a recall could mean their company’s bankruptcy. The stakes for biomedical device designers, especially in terms of safety, are incredibly high.
“That’s the main difference between medical device engineering and many other types of engineering,” said Jim Thompson, Siemens director of industry strategy for the medical device and pharmaceutical industries. “Medical device engineers not only need to design a really good product, but they need to think broadly about the safety and performance of the device. They need to come up with a high quality, high functioning device.”
Even if the challenges, such as strict U.S. Food and Drug Administration regulatory requirements and detailed development reporting process, are great, the potential rewards keep medical device companies investing in new products. The medical device industry is expected to reach an estimated $343 billion by 2021 with an expected compound annual growth rate of 4.6 percent, according to a September 2016 report from Lucintel, a materials and manufacturing market research firm in Irving, Texas.
To meet design requirements, engineers who work for medical device makers have been putting advanced modeling, simulation, and analysis software to use in innovative ways, such as creating models of the human anatomy that can be used to virtually test potential medical technologies. They have also put new tools such as 3-D printers to work building model prototypes for real-world testing.
;custompagebreak;Quantum Leap
Underlining the new importance of computer-aided design and virtual prototypes, FDA Commissioner Scott Gottlieb announced in July 2017 that the agency plans to integrate virtual testing and computer modeling into the medical device regulatory approval process. Allowing virtual testing to stand in for some real-world prototyping would help reduce costs and speed-up the regulatory approval process, Gottlieb said.
The move is a nod to the understanding that medical device engineers can more precisely evaluate their product’s function and performance using these technologies than with physical tests alone, said Tina Morrison, chair of the FDA’s modeling and simulation working group.
The models and simulations—in essence the virtual device and computerized proof it can operate in different conditions—help demonstrate the product's safety and efficacy, Morrison said. Simulations can also demonstrate the conditions under which the product might fail, which is needed for approval, she added.
Of course, most biomedical product development takes place within a 3-D design and analysis space before a physical prototype is ever built. The admission of more of this type of 3-D documentation means less physical testing needs to be done, said Arieh Halpern, director of global life science at engineering software maker Dassault Systèmes of Paris.
“If you look from development perspective, simulation and modeling is the next big quantum leap in product design acceleration in terms of animal studies and clinical trials,” Halpern said. “They let you run accelerated tests on different types of models to see how your design performs and to make changes.”
The FDA approval submissions for most devices require data from animal, bench, computational, and human tests to demonstrate a reasonable assurance of safety and effectiveness, Halpern said.
“Say you’re looking at the impact of a certain type of implanted device,” he said. “Instead of needing 200 patients to run a clinical trial, maybe you only need 50 if the FDA deems submittable the simulation data you used to model different types of conditions.”
To see how simulations could save time for design engineers, consider a new prosthetic device. Engineers could first design the device to function for a male of a specific height and weight. Then, using simulations of a virtual human, they could vary the height and weight to determine how the device would operate under those changed conditions. As they continued to change parameters, the engineers would measure device performance, and make changes to their design based on what they’d discovered.
After these virtual tests were complete, researchers could move to benchtop tests (testing a real-life prototype), and animal and human trials.
The FDA’s Center for Devices and Radiological Health is now creating a simulated human capable of serving as an in silico guinea pig. The center is building a library of computer regulatory testing models and a family of “virtual patients” for product design and testing.
The Living Heart Project is a similar effort in which researchers created a 3-D model of the human heart.
“An unlimited number of tests of a new design can be carried out simultaneously on the simulated heart rather than one at a time,” said Jean Colombel, Dassault Systèmes’s general manager of life sciences. The project is a collaboration among the FDA, Dassault Systèmes, and dozens of medical researchers, device manufacturers, and clinicians.
The model exists on the Dassault Systèmes 3DExperience platform. Engineers can access it and run virtual tests via the cloud, Colombel said, or they can use it as the foundation of their own computer models to perform specific types of virtual tests.
In September 2017, for example, Stanford University researchers unveiled their own additions to the Living Heart. Pharmaceutical companies can use that Stanford-created model to ensure the drugs they’re developing don’t induce cardiac arrhythmia, a potentially fatal irregular heartbeat, said Francisco Sahli, a Stanford mechanical engineering doctoral student who worked on the specialized model.
Currently, testing for the risk of cardiac arrhythmias can take years and involves costly animal and human studies, Sahli said.
To allow virtual testing, the Stanford team added detailed cellular models to the Living Heart and gave testers the ability to differentiate cell types within heart tissue. Those running the tests can also measure the model’s beating heart via electro-cardiogram.
Simulation and modeling tools also help engineers find the best design for their medical devices.
Engineers at Eli Lilly working with the company’s KwikPen and Trulicity needle-based insulin delivery devices had to determine whether they were compatible with a tapered needle from a third-party supplier.
It wasn’t a straightforward task.
“You have two considerations when you’re designing these,” said Bernard McGarvey, an engineering fellow at Eli Lilly. “You’re trying to force liquid through a needle, so you’d like to use a needle with a bigger inside diameter so you’d use less force to push the medicine through.
“But you also have to worry about the patient experience,” he said. “Imagine trying to puncture your skin with a 1-millimeter needle. The smaller the needle, the less the pain to a patient.”
Lilly engineers used COMSOL Multiphysics modeling software to simulate what would happen to the fluid within a potential supplier’s tapered needle when a patient exerted enough force to deliver the shot.
The models found the proposed needle could cut the required delivery force by 40 to 50 percent and deliver the correct amount of insulin while still tapering to a fine point, McGarvey said.
Individualized Devices
Other design concerns arise when manufacturers turn to additive manufacturing, a technology that enables companies to produce parts made from a variety of metals and plastics in very specific, even never-before-seen, shapes that are customized to particular users.
“When engineers have historically designed products they’d think about the functional and safety requirements and then come up with the shape,” Siemens’s Thompson said. “But they’d always be at least a little constrained by traditional manufacturing processes: injection molding, machining, stamping, forming, and folding. If you couldn’t make it that way, it’d be off the table.”
Objects produced via additive manufacturing needn’t hew to the traditional shapes made by the usual manufacturing methods.
“That’s important for biomedical design because there’s always been this need for organic shapes to fit within the human body, which is, by definition, organic,” Thompson said. “If engineers are able to take organic shape into account and they have a manufacturing method that works with even a lot size of one—why can’t they design products specifically tailored to the patient’s body?”
Those days are coming, said Gaurav Manchanda, vice president of market development at Formlabs, which makes 3-D printers used in healthcare and other industries.
Hospital systems, medical schools, and even doctors’ offices use the printers to produce physical models specific to an individual’s anatomy. Surgeons use the printed medical models to plan the patient’s surgery, Manchanda said.
To get those specialized models, images of the pertinent part of a patient’s anatomy—taken from magnetic resonance images and computer tomography scans—are cleaned up and turned into printable files with software from Formlab’s partner company Materialise.
“So instead of looking at a 2-D film and trying to visualize what’s happening inside the body, these 3-D medical models make a huge difference in saving time before the procedure,” Manchanda said.
“As the complexity goes up, the benefits go up as well,” he added. “If you have a pediatric heart patient, for instance, two surgeons can be looking at the same model as they prep and plan the surgery. Or in the operating room, a scrub nurse might hold up the model for the surgeon to use as reference during the operation.”
DJO Surgical, which makes hip, knee, elbow, and other implants started using a Formlab desktop printer in May 2017. Eight months later, the Austin, Texas, company had seen prototyping costs drop by 64 percent even as prototyping rate increased by four-and-a-half times, said Alex Drew, a DJO project mechanical engineer.
The company had been using a service bureau to print prototypes, but the process was expensive and could take more than a week, so engineers used the option sparingly, Drew said. In light of those drawbacks it “seemed silly not to have a 3-D printer in house,” he said.
Now engineers get immediate feedback from surgeons who implant 3-D prototypes in the company’s on-site operating room. The surgeon carries out the operation “from beginning to end” on a cadaver and then answer what Drew called a laundry list of engineering questions. The cycle is so fast that when surgeons offer suggestions after a morning operation, engineers can redesign the implant and print it up that afternoon, ready for another trial operation the next morning.
Recently DJO engineers came up with a unique way to study how a hip-implant prototype was positioned within the body.
“In some cases, you might need the implant to make contact with a specific part of the anatomy and you’d use an X-ray to look at fit,” Drew said.
The engineers designed the implant to leave interior passageways when printed. Those channels can be laced with wires that show up more clearly on an x-ray than the implant itself.
DJO Surgical engineers also used 3-D printed parts to run packaging tests to ensure implants remain safe and sterile during shipment on rolling, bouncing trucks. “We’re looking to see if the packaging develops holes, if the seals on the inner packaging that’s keeping the parts sterile stay intact over time,” Drew said.
A recent transportation test would have called for engineers to source 300 parts to run the tests. Finding and ordering those parts would have added months to the project. Instead, engineers printed many of the needed parts within three weeks and moved forward with testing, Drew said.
He estimates that eight months and 425 jobs after bringing in the printer DJO saved $60,000 in prototyping costs.
The DJO story points to the kinds of cost savings and competitive edge medical device designers gain when they incorporate new technologies into testing and validation.
“Speed to market is shortened because time can be saved on some of the testing,” said J. Freddy Hansen, staff research physicist at Abbott Labs in Pleasanton, Calif. “It’s also worth mentioning that with recent changes to the U.S. patent law, which is now first-to-file instead of first-to-invent, simulation can also speed up the intellectual property process, which is another competitive advantage.”
And, as the FDA’s embrace of modeling and simulation shows, today’s new engineering technology may be a regular part of tomorrow’s FDA approval submission.
Jean Thilmany is a technology writer based in St. Paul, Minn.
SIDEBAR: Better Modeling of Heat Treatment Doctors have increasingly turned to heat treatments to kill dangerous tissues, such as cancers. In the last 20 years, this treatment, also called ablation, has delivered the heat typically via an alternating electric current travelling along a catheter at radio frequencies (350–500 kHz).
A new technique promises to deliver the heat with greater precision and control by increasing the frequency of the current to 300 MHz to 300 GHz. This microwave ablation technology is now commercially available.
"The challenge is to monitor the ablation performance in real-time," said Casey Ladtkow, principal engineer in the Early Technologies unit of Medtronic's Minimally Invasive Therapies Group. "When performing ablations, physicians don't have continuous real-time feedback on the effectiveness of their procedure. If they could know exactly what is happening in real-time from start to finish, the effectiveness of ablation treatment would increase," he said.
Ladtkow and his team are using COMSOL Multiphysics software to develop new ablation probes in order to increase performance predictability and effectiveness. One development-stage project involves incorporating radiometers into the ablation probes in order to provide real-time data. That will enable a clinician to fine-tune the zone as needed during the procedure, and to make sure the heating effect destroys the targeted tissues while minimizing effects on the surrounding healthy tissue.
The team uses multiphysics simulation to better understand and optimize the properties of the probes. "The performance and accuracy of microwave ablation systems are affected by a number of dynamic factors that arise simultaneously in multiple physics domains," Ladtkow said. Simulating coupled thermal and electromagnetic effects around the radiative probe hardware helped the team determine radiometric performance under different conditions.
"Based on our simulations, we are now realizing the potential to introduce ablation devices that will allow clinicians to not only deliver a precise energy dose, but also monitor ablations in real time," Ladtkow said. "Multiphysics simulation enabled the rapid development, evaluation, and optimization of our design, which would not have been possible otherwise."
Learn more about advances in modeling and simulation in our Special Report
Read the latest issue of theMechanical Engineering Magazine.
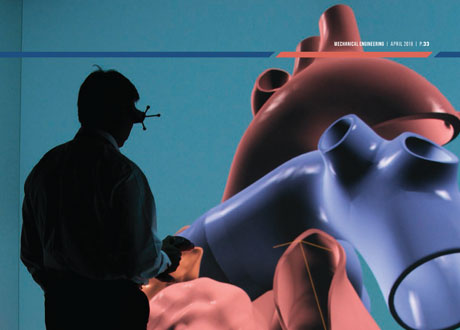
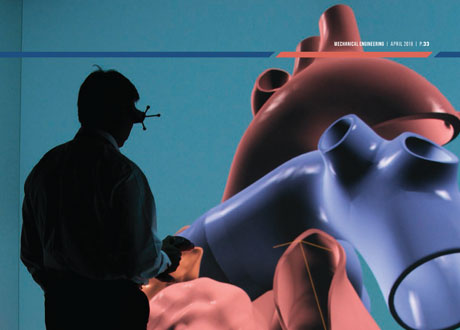