Regime Change in 3D Printed Microfluidics
Regime Change in 3D Printed Microfluidics
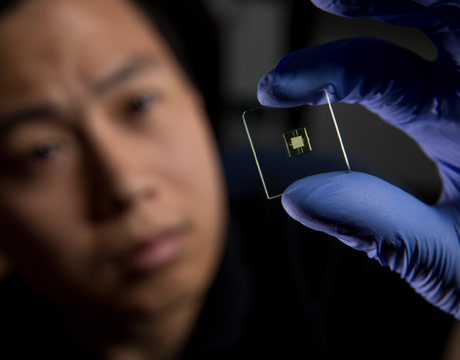
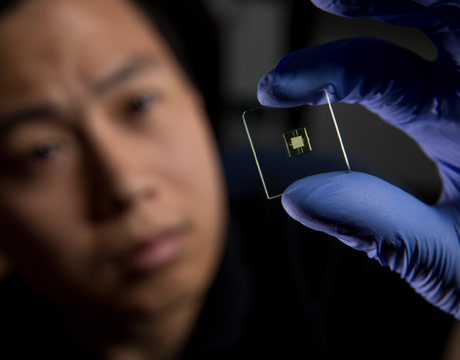
PhD student Hua Gong looks at a 3D-printed microfluidic device. Image: Brigham Young University
Engineers and chemists from Brigham Young University, Provo, UT, have broken through a major barrier to the wider use of 3D-printed microfluidics. With a customized printer and resin, the researchers were the first to fabricate a lab-on-a-chip with 3D-printed features small enough to fall within the “true” microfluidic regime below 100 micrometers. With fluidic flow channel cross sections of 18 x 20-micrometers, these devices could signal the arrival of 3D printing as a viable alternative to standard soft lithography or hot embossing for microdevice fabrication.
“Others have 3D-printed fluidic channels,” saysGregory Nordin, professor of electrical and computer engineering at BYU.“But they haven’t been able to make them small enough for microfluidics. So we decided to make our own 3D printer and research a resin that could do it.”
To those accustomed to viewing the world on the macro scale, the difference of a few micrometers may seem hair-splittingly small. But to microdevice engineers it’s a very big deal. By giving researchers easier, more affordable access to microdevices in the same size regime as complex commercially made devices, 3D printing could propel microfluidics to new heights in mainstream biological research and clinical diagnostics. “We’re deliberately trying to start a revolution in how microfluidic devices are fabricated,” Nordin says.
Microfluidics: What’s the Big Deal?
Microfluidic devices incorporate all steps in the biological measurement process – from sample pretreatment and preparation to detection – onto a small chip. Microscopic channels within the devices are on the ideal scale to study miniscule biological phenomena like disease markers, cells, and sub-cellular structures in blood or other body fluids. They make it possible to extract data from extremely small sample volumes with minimal amounts of reagent. The demand for microfluidics is growing rapidly in pharmaceutical and biotechnology research, where they are widely used in proteomics, high-throughput DNA sequencing, gene analysis, and enzymatic assays.
Early microfluidics were fabricated from silicon and glass using clean-room techniques and facilities already in use for semiconductor manufacturing. Glass-based chips were well suited for the prevailing electrophoretic measurement methods of the time, but with drawbacks. Both glass and silicon are brittle, and silicon is opaque to UV and visible light, making it incompatible with optical microscopes. The advent in the 1990s of soft lithography using elastomeric materials like polydimethylsiloxane (PDMS) dramatically advanced the field, but still requires equipment and expertise not typically available in life science labs. As an increasingly commonplace laboratory technology, 3D printing is eyed as the key to bringing microfluidics into the mainstream.
Interest in 3D printing has been steadily growing among lab-on-a-chip designers working to reduce the cost and complexity of prototyping and developing miniature devices that significantly outperform their full-scale counterparts. Some of the more intriguing emerging applications of microfluidics include precision drug delivery and sophisticated screening and diagnostic measurements for point-of-care (POC) use, particularly in the global health arena. In principle, robust 3D-printed microfluidics could make these mass-market clinical tools cheaper and easier to deploy in resource-scarce settings beset by infectious diseases.
As the technology matures, digital light processing stereolithography (DLP-SLA) has become a popular printing approach for lab-on-a-chip devices. DLP-SLA printers incorporate a micromirror array chip akin to those used in consumer projectors to create an optical pattern for each layer of a device during the printing process. However, Nordin’s team at BYU found that commercial DLP-SLA printers and materials are not capable of producing device features in the sub-100-micrometer regime – the sweet spot for measuring cellular and sub-cellular structures and processes. The minimum channel size attainable with conventional DLP-SLA printers appears to be in the 100-500 micrometer regime, although sizes in the millimeter and sub-millimeter range are more common, Nordin says. For 3D printing to offer a practical alternative to conventional fabrication techniques, sub-100-micrometer fabrication capabilities will be essential.
Equipment and Materials
The BYU system comprises a high-resolution light engine from Visitec, Lier, Norway; a 45-degree turning mirror with three axes of adjustment; a heavily modified DLP-SLA 3D printing mechanism from Solus, Junction 3D, Santa Clarita, CA; and custom-made mounts. The custom light engine and light source combination was critical to achieving the small in-plane (x-y) void size they sought while enabling them to work with a wider selection of materials for custom resin formulations. Having achieved unsatisfactory results using a 405-nm light emitting diode (LED) source, they instead used a 385-nm LED which dramatically expanded their options.
To formulate the ideal resin material for printing microfluidic void sizes, the team first developed a mathematical model for calculating the optical penetration depth and critical exposure time of a particular resin based on factors such as measured molar absorptivity and desired absorber concentrations. For the resin itself, they chose to start with a base of poly(ethylene glycol) diacrylate (PEGDA) monomer, which has low non-specific adsorption, is suitable for electrophoretic separations, is biocompatible, and can tolerate common solvents such as acetone and toluene. The PEGDA base is then mixed with a powered UV absorbing chemical that triggers the photopolymerization process in DLP-SLA printing.
A key challenge was to select a UV absorber with optical properties that would yield sufficiently small void sizes in the z dimension. The group subjected 20 potential compounds to a battery of tests to measure criteria such as solubility in PEGDA, optical absorption, hardness, Young’s modulus, fluorescence, and minimum achievable channel size. Ultimately, their mathematical model led them to select 3% 2-nitrophenyl phenyl sulfide (NPS) resin as the best choice to produce the minimum achievable void sizes. This approach was used to produce flow channels with a design height of 18 micrometers and an initial channel width of 38 micrometers. To further reduce the width, a novel channel narrowing technique was developed to achieve physical widths of approximately 20 micrometers. The process was used to fabricate 41-mm-long 3D serpentine flow channels with high-aspect-ratio flow channels on a 0.12-mm squared chip. The team’s serpentine design takes about 30 minutes to print. Multiple devices can be batched in the same print job to increasing throughput and economic efficiency.
The team is now putting their new biochip to work in experiments designed to demonstrate their suitability in real-world diagnostic applications. For example, co-investigator Adam Woolley – a chemist – is exploring their use to detect biomarkers that predict a pregnant woman’s risk for preterm childbirth. Worldwide, 15 million babies are born prematurely each year and face a wide range of complications that, collectively, are the leading cause of death of children under five years old. Those who survive face a lifetime of heightened risk of chronic diseases that, in the United States alone, cost society more than $30 billion a year to treat. With early intervention, preterm births can often be forestalled but, to date, there is no biomarker-based diagnostic to confirm a woman’s risk.
Woolley has already developed conventional chips fabricated with hot embossing that can screen for nine preterm-birth biomarkers in a tiny drop of the mother’s blood with 90% accuracy. Woolley and Nordin are seeking National Institutes of Health support for research that would adapt their 3D printing method to this application. Thus, in the case of 3D printed microfluidics, the next “killer app” may in fact turn out to be a life-saver.
Michael MacRae is an independent writer.
We’re deliberately trying to start a revolution in how microfluidic devices are fabricated.Prof. Gregory P. Nordin, Brigham Young University
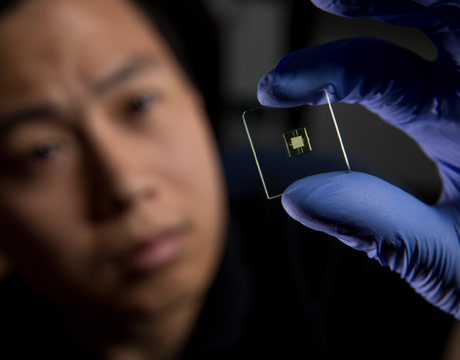
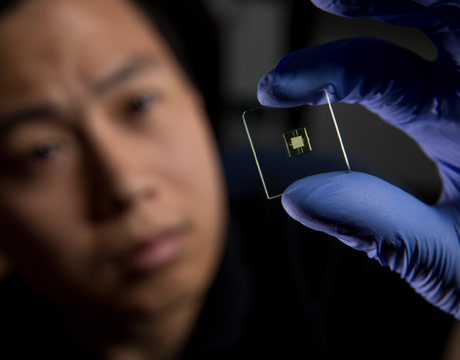