AM Needs MEs
AM Needs MEs
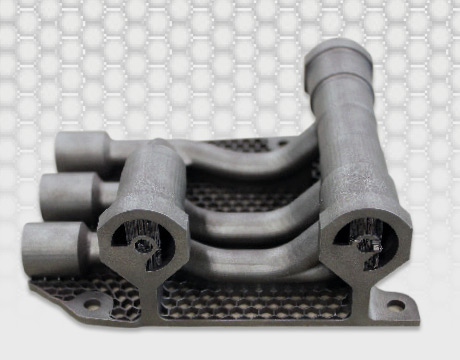
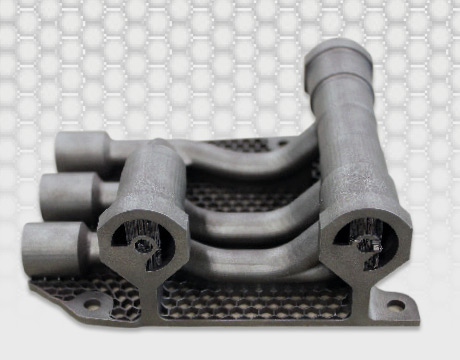
Wrench flats and machining guides are 3-D printed in a part as aids to post-processing and assembly. Image: Penn State CIMP-3D
When I started working in additive manufacturing three years ago, I thought 3-D printing of metals would be easy because I had worked with 3-D printed plastics for nearly two decades. I could not have been more wrong. AM is rewriting the rules of how we design, make, and qualify parts, and 3-D metal printing needs all the help it can get from mechanical engineers.
For starters, mechanical engineers have traditionally designed parts by selecting a material with the best known properties (based on how it was processed and heat treated) and then creating the shape they want.
With additive manufacturing, the process is reversed. We print the shape that we want, and then we relieve stress, heat treat, age, or anneal the part until we get the material we want.
The process sounds simple, but it is not. The thermal cycling that 3-D printed metallic parts see during the layer-by-layer melting and fusion process, be it by laser or electron beam, has a huge influence on the microstructure, which in turn affects the material properties. So, the part that we thought we had designed and engineered for specific strength and mechanical properties is not necessarily the part we get—or it may be, depending on how it was made and post-processed. Because there are no good models to predict any of this, companies are currently treating each part as a "one off," which equates to extensive testing and verification for each part made by additive manufacturing, which is neither cost effective nor an efficient use of resources.
So why should we, as mechanical engineers, care about this? The answer's simple: every step of the process has numerous unknowns right now, and the tools, methods, and fundamental understanding needed to answer these questions do not exist. In short, MEs have a lot of work to do to help additive manufacturing reach its full potential.
Even though manufacturers have been selling powder-bed fusion and directed energy deposition systems for several years, we still do not really know exactly what is going on in these machines as the parts are being made. Modeling laser-powder interactions is difficult, especially since the physics and heat transfer phenomena are not fully understood in AM systems, particularly powder-bed fusion systems.
The models and simulations that have been created are computationally expensive and still undergoing validation and verification. Few can simulate a full part through its entire build process. Engineers trying to model and simulate 3-D metal printing using existing finite element analysis packages, such as Nastran or Abaqus, need billions of elements and billions of time steps, which invariably crash the software for even simple part geometries.
Even if you can predict the thermal history the part experiences during a build, that is only half the problem. Models are needed to predict the residual stresses that will result and distortions that will occur, and estimate what the resulting microstructure is going to be—all of which will change for different process parameter settings, build orientations, and metallic alloys and powder parameters including particle size, distribution, and morphology.
Finally, because these AM processes are not well understood, we do not have any good tools for designing build supports in powder bed systems that can "anchor" the part to the build plate and counteract the thermal stresses that develop as the part is built up layer by layer. Based on our experience in the CIMP-3D lab at Penn State, at least 80 percent of build failures in powder bed fusion systems result from poorly designed support structures, yet analytical tools to optimize supports and corresponding build orientation of the part are limited at best. Polymer 3-D printing systems can use supports to counteract gravity and to ensure a successful build, but polymer supports are water soluble and easy to remove. Not so when 3-D printing metals—supports anchoring the part to the build plate must be removed by cutting, grinding, and other labor-intensive processes.
Parts want to curl up (like a potato chip) during a build and have been known to tear themselves from the build plate, particularly titanium parts fabricated using laser-based powder-bed fusion. There are ways to overcome this, but the process requires a lot of trial and error right now, which is expensive and time-consuming.
;custompagebreak;Because we do not have a complete understanding of what is going on inside an AM system as a part is being fabricated, design guidelines and design rules for AM are not readily available, or are nascent at best. Studies are starting to become available, for example, to understand what overhangs, wall thicknesses, and geometries can be easily built with (or without) supports. But these values vary by material (e.g., Ti64 vs. IN718) and by machine (e.g., an EOS system vs. an Arcam system).
Private companies are spending millions of dollars of their own R&D funding to create AM knowledge bases. Little of that knowledge, however, is being shared because it provides a competitive advantage for those companies that have it. Since anyone can buy an AM system, the real power lies in knowing how to use it. But everyone will benefi t if we collaborate and utilize resources like America Makes, the National Additive Manufacturing Innovation Institute, to share information and advance AM.
Engineering lightweight structures, designing sophisticated internal cooling passageways, or combining multi-part assemblies into a single printed component are just some of the benefits touted for additive manufacturing. Without the design rules, though, we do not have good computer-aided design tools to achieve those ends.
Like 3-D printing technology, topology-optimization tools, for instance, have been around for many decades, yet we only now have the means to fabricate the intricate and organic shapes that provide optimal loading for minimum weight structures. GE's jet engine bracket challenge was a great example of how AM can be used to lightweight components.
GE posted the design specifications and loading conditions for one of its jet engine brackets and crowd-sourced ideas to reduce its weight (https://grabcad.com/challenges/ge-jet-enginebracket-challenge). Nearly 700 entries from more than 50 countries were submitted within a few months, and the top ten designs were identifi ed, 3-D printed, and then tested. The winning bracket, designed by M Arie Kurniawan from Salatiga, Indonesia, weighed 0.72 pound, nearly 84 percent lighter than the original 4.48-pound bracket made using subtractive manufacturing processes (http://www.gereports.com/post/77131235083/jet-enginebracket-from-indonesia-wins-3dprinting).
Making the part using additive manufacturing is not straightforward either. A lot can go wrong during the build process. The 3dprintingindustry.com blog carried a three-part entry called, "3D Printing Titanium & the Bin of Broken Dreams." In Part 3, Spencer Wright, a design expert at the organizational consultancy Undercurrent, describes six build failures, which he attributes to various causes, including the stripped-down simplicity of STL fi les and manufacturing tolerances too generous for larger parts. Mechanical engineers are salivating at the potential to put any material they want at any position they want in three-dimensional space with additive manufacturing to optimize its performance,
;custompagebreak;but the design tools and analyses do not yet exist—more opportunities for mechanical engineers to help AM. The interactions between how you design a part and how you build it in an AM system are tightly coupled, yet not well understood. Engineers and designers are used to working with "design allowables" for materials made by known processes (such as casting, forging, and machining), but those design allowables do not yet exist for AM, nor do the "Design for Additive Manufacturing" guidelines that engineers need to successfully design parts for AM fabrication. A forthcoming issue of ASME's Journal of Mechanical Design is gathering the current state of best practices, which are far more advanced for polymers than they are for metals.
Even if we can solve the design and material issues, manufacturers are still hesitant to fully embrace the technology. High-end AM systems are still pricey (more than $500,000 in many cases), and machine operation and maintenance are costly. Maintenance agreements can run upwards of $50,000 per year for some systems, putting them well out of reach of many small and mid-size enterprises.
The materials are also extremely expensive. This is particularly important for powder-bed systems as the build height defines the volume of powder needed. We once needed $5,000 of powder to build $200 of parts due to a tall part that we had designed, a mistake we do not want to repeat.
Meanwhile, many AM systems lack the monitoring and sensing capabilities needed to control the processes, making it difficult to qualify equipment for production. It also makes it difficult to determine when and why a defect occurred if the process cannot be monitored. Mechanical engineers with expertise in sensing and controls have a wide-open playing field in this area.
Heat treatment schedules and post-processing considerations for AM-fabricated parts have received little attention to date from the broader mechanical engineering community, yet are crucial for achieving a functional AM part. The question about whether or not to apply hot isostatic pressing (HIP) to a 3-D printed part remains unanswered. Some manufacturers use HIP for all their AM parts to improve fatigue strength and reduce porosity, while others feel it is not needed or too expensive to use on every AM part.
While HIP may reduce internal porosity in some printed parts, it does little to the exterior surface finish, which can vary considerably based on build orientation and material. Identical parts built in the same orientation using two different materials will have two different surface finishes.
This three-way interaction among part features, build orientation, and material choice is difficult enough as it is, but the extent of the interaction also varies by machine. A laser powder-bed fusion system will produce a very different part from that of an electron beam-based powder-bed system, everything else being equal.
Finally, even though we can now realize complex internal features and passageways for conformal cooling and improved heat transfer, how do we know they printed as we designed them? We can't see them to inspect them; so, new techniques for non-destructive inspection are needed to help certify parts. While X-ray computed tomography (CT) scanning shows promise, part size and material composition limit what this technology can do. CT scanning systems are still rather expensive, and companies certainly do not want to scan every part that they produce using AM.
We need mechanical engineers to help rethink and develop new technology for inspection and certification of 3-D printed parts without having to resort to printing 10 parts, testing nine of them, and keeping the last one for use, hoping it is as good as the ones we tested.
The issues and challenges are far too numerous for one person or team to solve, and so we hope that more MEs will not dismiss AM outright as a new fad for 3-D printing and instead will talk to their colleagues in materials science, metallurgy, industrial engineering, and other fields to see what they can do to help. Additive manufacturing is poised to become as pervasive as computers—used almost anywhere for almost anything. At home, if a handle or connector breaks, then we will just print a new one. At work, if we want to verify the fit and feel of a part that we designed, then we will just make one on our desktop 3-D printer; we won't have to wait anymore for the prototype shop to make it.
At school, we are already starting see a huge shift in how we integrate 3-D printing into the classroom. Entering freshmen are clamoring to access and use 3-D printing as soon as they come to campus; they do not want to wait until their senior year to use 3-D printers on their capstone design projects. These students have had easy access to these capabilities in middle and high schools, and many mechanical engineering students are bringing (or building) their own 3-D printers and running them in their dorms and apartments. It may not be long before mechanical engineering students may be required to bring their own laptop and 3-D printer to campus their freshmen year.
Most mechanical engineering curricula have not yet integrated 3-D printing and additive manufacturing into the classroom. We have a huge opportunity to reinvent how we teach and train mechanical engineers if we embrace AM.
It's all there, and it's never been more affordable and within reach. Low-end polymer 3-D printers now cost about the same as the original 2-D laser printers (which simply drew images on paper) did when they first came out. Prices of metallic AM systems are starting to drop as the field gets more competitive and patents expire, and everyone is trying to reduce material costs and offer a wider palette of materials to 3-D print.
We just have to be willing, as mechanical engineers and educators training the next generation of mechanical engineers, to look beyond the hype to understand what's real, identify the possibilities, and help advance AM technology and education. Only then will additive manufacturing realize its full potential and help revitalize manufacturing in the United States.
Our K-12 students are already doing it. Will we be ready when they go to college and enter the workforce?
Timothy W. Simpson is a professor of mechanical and industrial engineering, and co-director of the Center for Innovative Materials Processing through Direct Digital Deposition (CIMP-3D) at the Pennsylvania State University.
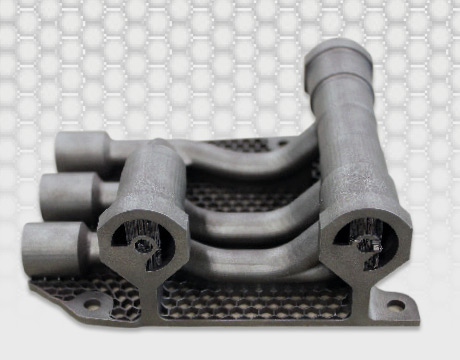
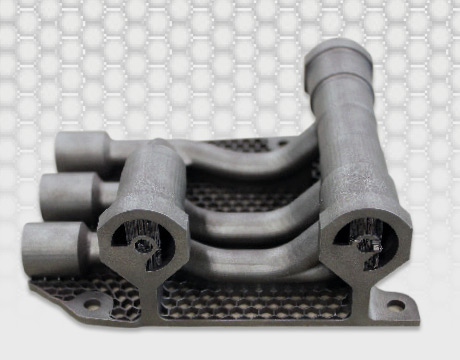